The Molecular Lung
How a single protein flaunts the limits of physics to behave like a molecular organ: An excerpt from Max Perutz's "The Second Secret of Life."
In 1937, Max Perutz set out to elucidate the structure of a protein that had already been the subject of intense biological interest for a century. He could have no idea what a complicated, incredible machine he had chosen to study — one that compresses the dynamic equilibria of an entire biological cycle into a single molecule; a molecular lung.
Brian
I recently ordered a copy of Max Perutz’s essay collection, I Wish I’d Made You Angry Earlier, hoping that the title promised a manifesto against politeness and respectful discourse in biology. Unfortunately, that is not the case. But the book still seems to offer a wealth of other noteworthy writings.
Following his breakthrough work on determining the structure and then molecular mechanism of hemoglobin — each of which separately required a mere two decades of work; pretty low-hanging fruit, obviously, but for some reason it won him a Nobel Prize — Perutz embarked on a second career of writing about scientific heavyweights and heavy science controversies in a lay-accessible manner. Included in his essay book, however, are 9 works somewhat apologetically directed toward other scientists. The first of which, it turns out, is a masterful discussion of his molecule, hemoglobin, which also appeared in the December, 1978 issue of Scientific American (under a less floral name).
That essay, “The Second Secret of Life” is a perfect biological lecture — and as such, it by rights ought to be available on the internet in text form. Thus, since I have a “blog,” I am using said blog to set matters right.
The reader is thus forewarned that the essay sampled here is a challenging read. But while Perutz provides only the minimum explanation necessary to convey the grandeur and genius of the system as a whole, he also does not abridge any aspect with reference to some assumed, external pre-existing subject-matter knowledge. His tour of the hemoglobin molecule is concise, but self-complete.
My excerpt covers Perutz’s discussion of the properties of the molecule that were understood before his elucidation of its structure. The complete essay, naturally is available via purchase of a copy of his book, or online access to the relevant issue of Scientific American.
Max Perutz
“The Second Secret of Life”
or
“Hemoglobin Structure and Respiratory Transport”
Why grasse is greene, or why our blood is red. Are mysteries which none have reach'd unto. In this low forme, poore soule, what wilt thou doe?
JOHN DONNE, Of the Progresse of the Soule
In 1937, I chose hemoglobin as the protein whose structure I wanted to solve, but it proved so much more complex than any solved before that it eluded me for more than 20 years. First success came in 1959, when Ann F. Cullis, Hilary Muirhead, Michael G. Rossmann, Tony C.T. North, and I first unraveled the architecture of the hemoglobin molecule in outline. We felt like explorers who have discovered a new continent, but it was not the end of the voyage, because our much-admired model did not reveal its inner workings—it provided no hint about the molecular mechanism of respiratory transport. Why not? Well-intentioned colleagues were quick to suggest that our hard-won structure was merely an artifact of crystallization and might be quite different from the structure of hemoglobin in its living environment, which is the red blood cell.
Hemoglobin is the vital protein that conveys oxygen from the lungs to the tissues and facilitates the return of carbon dioxide from the tissues back to the lungs. These functions and their subtle interplay also make hemoglobin one of the most interesting proteins to —
Brian
Whoa! I almost forgot to set the mood! When the modern science-thinker has heavy science-thinking to do, after all, the other party must set the mood. And there is no better stimulator of the virile, lusty Spirit of Discovery than a pairing of 70s-vintage Scientific American ad-spreads.
Ah, that’s better! Ok. Spirit of Discovery = Aroused, cow-person! Now, where were we?
Max Perutz
Hemoglobin is the vital protein that conveys oxygen from the lungs to the tissues and facilitates the return of carbon dioxide from the tissues back to the lungs. These functions and their subtle interplay also make hemoglobin one of the most interesting proteins to study. Like all proteins, it is made of amino acids strung together in a polypeptide chain. The amino acids are of 20 different kinds and their sequence in the chain is genetically determined. A hemoglobin molecule is made up of four polypeptide chains, two alpha chains of 141 amino acid residues each and two beta chains of 146 residues each. The alpha and beta chains have different sequences of amino acids but fold up to form similar three-dimensional structures. Each chain harbors one heme, which gives blood its red color. The heme consists of a ring of carbon, nitrogen, and hydrogen atoms called porphyrin, with an atom of iron, like a jewel, at its center. A single polypeptide chain combined with a single heme is called a subunit of hemoglobin or a monomer of the molecule. In the complete molecule four subunits are closely joined, as in a three-dimensional jigsaw puzzle, to form a tetramer.
Hemoglobin Function
In red muscle there is another protein, called myoglobin, similar in constitution and structure to a beta unit of hemoglobin but made up of only one polypeptide chain and one heme. Myoglobin combines with the oxygen released by red cells, stores it, and transports it to the subcellular organelles called mitochondria, where the oxygen generates chemical energy by the combustion of glucose to carbon dioxide and water. Myoglobin was the first protein whose three-dimensional structure was determined—the structure was solved by my colleague John C. Kendrew and his collaborators.
Myoglobin is the simpler of the two molecules. This protein, with its 2,500 atoms of carbon, nitrogen, oxygen, hydrogen, and sulfur, exists for the sole purpose of allowing its single atom of iron to form a loose chemical bond with a molecule of oxygen (O2). Why does Nature go to so much trouble to accomplish what is apparently such a simple task? Like most compounds of iron, heme by itself combines with oxygen so firmly that its bond with an oxygen atom, once formed, is hard to break. This happens because an iron atom can exist in two states of valency: ferrous iron, carrying two positive charges, as in iron sulfate, which anemic people are told to eat, and ferric iron, carrying three positive charges, as in iron oxide, or rust. Normally, ferrous heme reacts with oxygen irreversibly to yield ferric heme, but when ferrous heme is embedded in the folds of the globin chain, it is protected so that its reaction with oxygen is reversible. The effect of the globin on the chemistry of the heme has been explained with the discovery that the irreversible oxidation of heme proceeds by way of an intermediate compound in which an oxygen molecule forms a bridge between the iron atoms of two hemes. In myoglobin and hemoglobin, the folds of the polypeptide chain prevent the formation of such a bridge by isolating each heme in a separate pocket. Moreover, in the protein the iron is linked to a nitrogen atom of the amino acid histidine, which donates negative charge that enables the iron to form a loose bond with oxygen.
An oxygen-free solution of myoglobin or hemoglobin is purple like venous blood, and when oxygen is bubbled through such a solution, it turns scarlet like arterial blood. If these proteins are to act as oxygen carriers, then hemoglobin must be capable of taking up oxygen in the lungs, where it is plentiful, and giving it up to myoglobin in the capillaries of muscle, where it is less plentiful: myoglobin in turn must pass the oxygen on to the mitochondria, where it is still scarcer.
A simple experiment shows that myoglobin and hemoglobin can accomplish this exchange because there is an equilibrium between free oxygen and oxygen bound to heme iron. Suppose a solution of myoglobin is placed in a vessel constructed so that a large volume of gas can be mixed with it and so that its color can also be measured through a spectroscope. Without oxygen only the purple color of deoxymyoglobin is observed. If a little oxygen is injected, some of the oxygen combines with some of the deoxymyoglobin to form oxymyoglobin, which is scarlet. The spectroscope measures the proportion of oxymyoglobin in the solution. The injection of oxygen and the spectroscopic measurements are repeated until all the myoglobin has turned scarlet. The results are plotted on a graph with the partial pressure of oxygen on the horizontal axis and the percentage of oxymyoglobin on the vertical axis. The graph has the shape of a rectangular hyperbola—it is steep at the start, when all the myoglobin molecules are free, and it flattens out at the end, when free myoglobin molecules have become so scarce that only a high pressure of oxygen can saturate them (Figure 1).
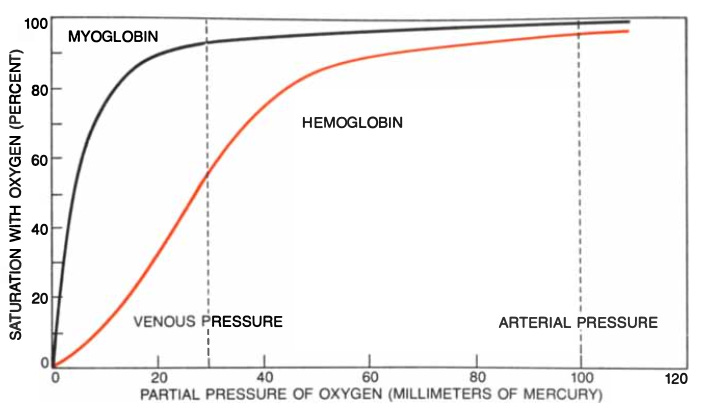
To understand this equilibrium one must visualize its dynamics. Under the influence of heat the molecules in the solution and in the gas are whizzing around erratically and are constantly colliding. Oxygen molecules are entering and leaving the solution, forming bonds with myoglobin molecules and breaking away from them. The number of iron-oxygen bonds that break in one second is proportional to the number of oxymyoglobin molecules. The number of bonds that form in one second is proportional to the frequency of collisions between myoglobin and oxygen, which is determined in turn by the product of their concentrations. When more oxygen is added to the gas, more oxygen molecules dissolve, collide with and bind to myoglobin. This raises the number of oxymyoglobin molecules present and therefore also the number of iron-oxygen bonds liable to break, until the number of myoglobin molecules combining with oxygen in one second becomes equal to the number that lose their oxygen in one second. When that happens, a chemical equilibrium has been established.
Brian
Perutz knocks this explanation of binding equilibrium out of the park. However, perhaps it is worthwhile to offer a redundant metaphor. The reader may imagine that there are 100 children in a room, and a certain number of camp counselors materialize in the room to offer them toys. The children who take up toys then drop them after a few minutes of play, at which point counselors pick them up and try to induce children to play with them again.
This dynamic is intrinsic to all soluble, reversible binding equilibriums: It will be easy to hand toys off when very few kids have toys, because the counselors trying to give away toys will mostly encounter toy-less kids. But once a majority of kids have toys, the efficiency of giving toys to kids begins to plummet; it becomes harder to give away toys. And if the kids are meanwhile easily bored, then it will take an extraordinary number of counselors (high counselor concentration / pressure) to get to either equilibrium (half of kids have toys, half don’t) or saturation.
But more importantly, the paradigm of “easy to donate toys at first, equilibrium, hard to donate toys later” is indifferent to the consideration of how strongly kids bind to toys. The saturation curve will be parabolic no matter what, because it is simply a mathematical reflection of the effect of already-having-toys on the ease of donating toys; and because already-having-toys is determined by how many counselors are trying to give out toys. Thus, it will always be easy to increase how many kids have toys if (relative to the equilibrium counselors concentration) few counselors are around; and hard to increase how many kids have toys if (relative to the equilibrium) loads of counselors are around.
And so — oops, wait, ad break
Oh, wow! Talk about juicing the Spirit of Discovery right up to eleven!
(Also — Polaroid made an auto-focusing camera?! I have never seen a single iteration of this incredible-looking machine in my life.
Anyway, back to the editor commentary… )
And so, it is this intrinsic dichotomy between easy and hard to donate a ligand in solution, that renders hemoglobin such an incredible flaunt-er of the limits of physics: In total defiance of the paradigm of any soluble, ligand-binding molecule, it becomes more likely to give up oxygen when it has less oxygen, and more likely to take up oxygen when it already has more. And it is this miraculous behavior which allows it to convey oxygen from a high pressure to low pressure environment and to shepherd CO2 in the other direction, enabling vertebrate life.
Now, let us resume our lecture.
Max Perutz
The equilibrium is best represented by a graph in which the logarithm of the ratio of oxymyoglobin molecules (Y) to deoxymyoglobin molecules (1-Y) is plotted against the logarithm of the partial pressure of oxygen. The hyperbola now becomes a straight line at 45 degrees to the axes. The intercept of the line with the horizontal axis drawn at Y/(1-Y) = 1 gives the equilibrium constant K. This is the partial pressure of oxygen at which exactly half of the myoglobin molecules have taken up oxygen. The greater the affinity of the protein for oxygen, the lower the pressure needed to achieve half-saturation and the smaller the equilibrium constant [thus, lower equilibrium constants describe tighter binding]. The 45° degree slope remains unchanged, but lower oxygen affinity shifts the line to the right [looser binding] and higher affinity shifts it to the left [higher binding] (Figure 2).
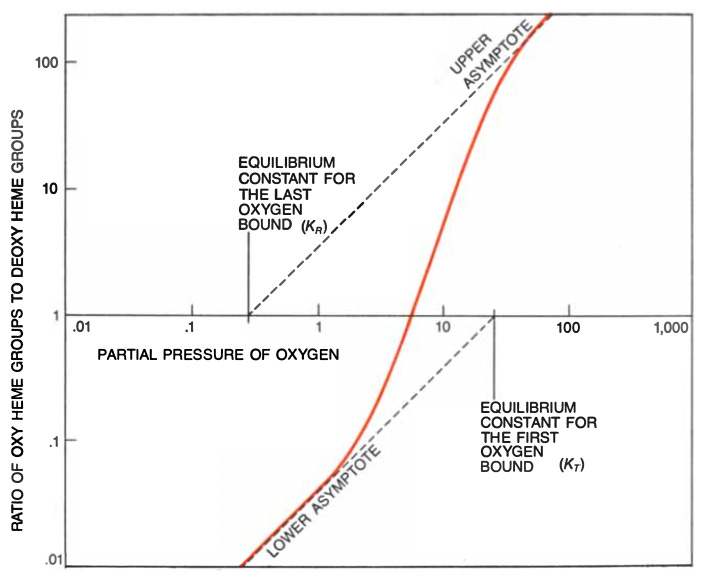
If the same experiment is done with blood or with a solution of hemoglobin, an entirely different result is obtained. The curve rises gently at first, then steepens and finally flattens out as it approaches the myoglobin curve. This strange sigmoid shape signifies that oxygen-free molecules (deoxyhemoglobin) are reluctant to take up the first oxygen molecule but that their appetite for oxygen grows with the eating. Conversely, the loss of oxygen by some of the hemes lowers the oxygen affinity of the remainder. The distribution of oxygen among the hemoglobin molecules in a solution therefore follows the biblical parable of the rich and the poor: "For unto every one that hath shall be given, and he shall have abundance: but from him that hath not shall be taken away even that which he hath." This phenomenon suggests some kind of communication between the hemes in each molecule, and physiologists have therefore called it heme-heme interaction.
In a logarithmic graph the equilibrium curve begins with a straight line at 45 degrees to the axes because oxygen molecules are so scarce at first that only one heme in each hemoglobin molecule has a chance of catching one of them, and all the hemes therefore react independently, as in myoglobin. As more oxygen flows in, the four hemes in each molecule begin to interact and the curve steepens. The tangent to its maximum slope is known as Hill's coefficient (n), after the physiologist A. V. Hill who first attempted a mathematical analysis of the oxygen equilibrium. The normal value of Hill's coefficient is about 3; without heme-heme interaction it becomes unity. The curve ends with another line at 45 degrees to the axes because oxygen has now become so abundant that only the last heme in each molecule is likely to be free, and all the hemes in the solution react independently once more (Figure 2).
Cooperative Effects
Hill's coefficient and the oxygen affinity of hemoglobin depend on the concentration of several chemical factors in the red blood cell: protons (hydrogen atoms without electrons, whose concentration can be measured as pH); carbon dioxide (CO2); chloride ions (C1-); and a compound of glyceric acid and phosphate called 2,3-diphosphoglycerate (DPG). Increasing the concentration of any of these factors shifts the oxygen equilibrium curve to the right, toward lower oxygen affinity, and makes it more sigmoid. Increased temperature also shifts the curve to the right, but it makes it less sigmoid. Strangely, none of these factors, with the exception of temperature, influences the oxygen equilibrium curve of myoglobin, even though the chemistry and structure of myoglobin are related closely to those of the individual chains of hemoglobin.
What is the purpose of these extraordinary effects? Why is it not good enough for the red cell to contain a simple oxygen carrier such as myoglobin? Such a carrier would not allow enough of the oxygen in the red cell to be unloaded to the tissues, nor would it allow enough carbon dioxide to be carried to the lungs by the blood plasma. The partial pressure of oxygen in the lungs is about 100 millimeters of mercury, which is sufficient to saturate hemoglobin with oxygen whether the equilibrium curve is sigmoid or hyperbolic. In venous blood, the pressure is about 35 millimeters of mercury; if the curve were hyperbolic, less than 10 percent of the oxygen carried would be released at that pressure, so a man would asphyxiate even if he breathed normally.
The more pronounced the sigmoid shape of the equilibrium curve is, the greater the fraction of oxygen that can be released. Several factors conspire to that purpose. Oxidation of nutrients by the tissues liberates lactic acid and carbonic acid; these acids in turn liberate protons, which shift the curve to the right, toward lower oxygen affinity, and make it more sigmoid. Another important regulator of the oxygen affinity is DPG. The number of DPG molecules in the red cell is about the same as the number of hemoglobin molecules, 280 million, and probably remains fairly constant during circulation. A shortage of oxygen, however, causes more DPG to be made, which helps to release more oxygen. With a typical sigmoid curve nearly half of the oxygen carried can be released to the tissues. The human fetus has a hemoglobin with the same alpha chains as the hemoglobin of the human adult but different beta chains, resulting in a lower affinity for DPG. This gives fetal hemoglobin a higher oxygen affinity and facilitates the transfer of oxygen from the maternal circulation to the fetal circulation.
Carbon monoxide (CO) combines with the heme iron at the same site as oxygen, but its affinity for that site is 150 times greater; carbon monoxide therefore displaces oxygen, which explains why it is so toxic. In heavy smokers, up to 20 percent of the oxygen combining sites can be blocked by carbon monoxide, so that less oxygen is carried by the blood. In addition carbon monoxide has an even more sinister effect. The combination of one of the four hemes in any hemoglobin molecule with carbon monoxide raises the oxygen affinity of the remaining three hemes by heme-heme interaction. The oxygen equilibrium curve is therefore shifted to the left, which diminishes the fraction of the oxygen carried that can be released to the tissues.
If protons lower the affinity of hemoglobin for oxygen, then the laws of action and reaction demand that oxygen lowers the affinity of hemoglobin for protons. Liberation of oxygen causes hemoglobin to combine with protons and vice versa; about two protons are taken up for every four molecules of oxygen released, and two protons are liberated again when four molecules of oxygen are taken up. This reciprocal action is known as the Bohr effect and is the key to the mechanism of carbon dioxide transport (Figure 3). The carbon dioxide released by respiring tissues is too insoluble to be transported as such, but it can be rendered more soluble by combining with water to form a bicarbonate ion and a proton. The chemical reaction is written:
CO2 plus H20←→HCO3- plus H+
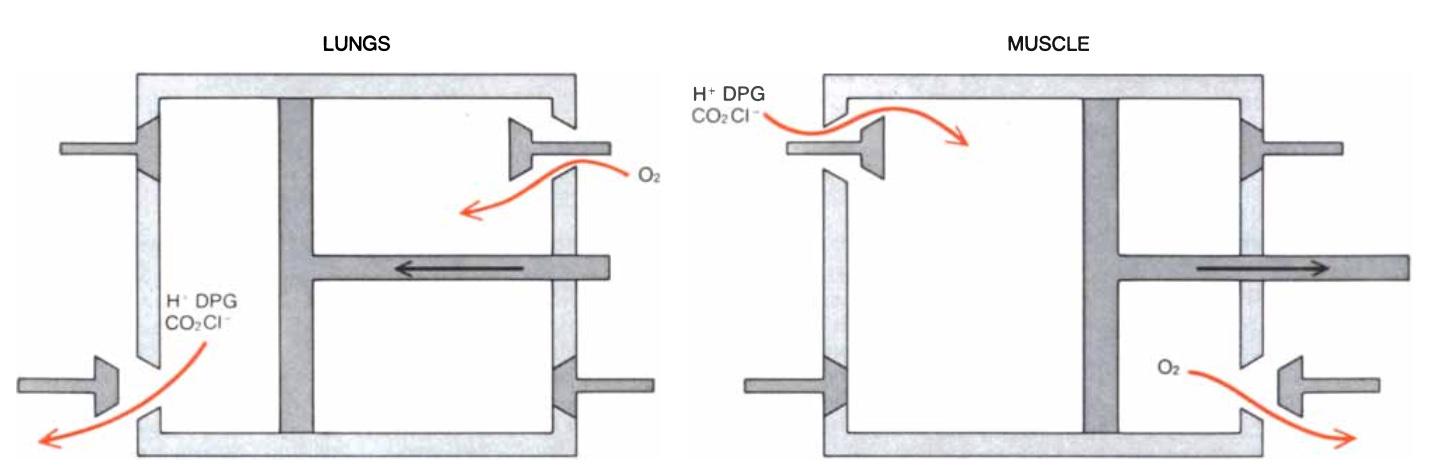
In the absence of hemoglobin this reaction would soon be brought to a halt by the excess of protons produced, like a fire going out when the chimney is blocked. Deoxyhemoglobin acts as a buffer, mopping up the protons and tipping the balance toward the formation of soluble bicarbonate. In the lungs the process is reversed. There, as oxygen binds to hemoglobin, protons are cast off, driving carbon dioxide out of solution so that it can be exhaled. The reaction between carbon dioxide and water is catalyzed by carbonic anhydrase, an enzyme in the red cells. The enzyme speeds up the reaction to a rate of about half a million molecules per second, one of the fastest of all known biological reactions.
There is a second, but less important, mechanism for transporting carbon dioxide. The gas binds more readily to deoxyhemoglobin than it does to oxyhemoglobin, so that it tends to be taken up when oxygen is liberated and cast off when oxygen is bound. The two mechanisms of carbon dioxide transport are antagonistic. For each molecule of carbon dioxide bound to deoxyhemoglobin either one or two protons are released, which oppose the conversion of other molecules of carbon dioxide to bicarbonate. Positively charged protons entering the red cell draw negatively charged chloride ions in with them, and these ions too are bound more readily by deoxyhemoglobin than by oxyhemoglobin. DPG is synthesized in the red cell itself and cannot leak out through the cell membrane. It is strongly bound by deoxyhemoglobin and only very weakly bound by oxyhemoglobin.
Heme-heme interaction and the interplay between oxygen and the other four ligands are known collectively as the cooperative effects of hemoglobin. Their discovery by a succession of physiologists and biochemists took more than half a century and aroused many controversies. In 1938, Felix Haurowitz of the Charles University in Prague made another vital observation. He discovered that deoxyhemoglobin and oxyhemoglobin form different crystals, as though they were different chemical substances, which implied that hemoglobin is not an oxygen tank but a molecular lung because it changes its structure every time it takes up oxygen or releases it.
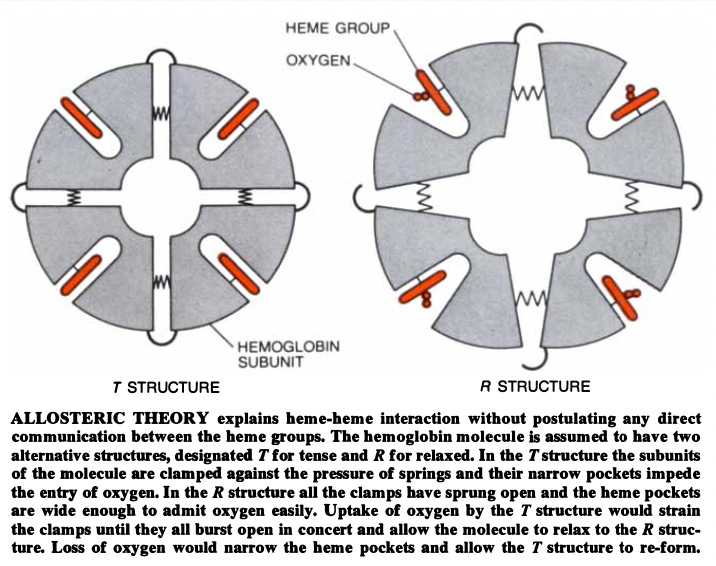
[End of excerpt.]
Thanks for the deep dive Brian. Now, let me introduce a molecule that should strike terror into everyone who has read your piece: Carboxyhemoglobin!!!!
Yes, that's what results when CO gets introduced into this elegantly balanced system. Deadly based on the very strong binding of CO vs either O2 or CO2.
Anyway, I also want to say that you attract the coolest group of anti-reductionist commentors here. Love the comments reflecting on the complexity of biological life... and my additional comment would be, based on the many years of experiments run by Dean Radin showing how our consciousness can to a measurable degree collapse the wave function via double slit-based monitoring, that this complexity extends far beyond the biology.
Finally, I have come up with a new way to describe IgG4 antibodies. NPC antibodies.
Life is amazing, when we begin to understand the intricate evolved complexity underlying its most basic functions.